Last time, we explored the work of Friedrich Miescher and his discovery of DNA, but we didn’t talk about how DNA stores information about the traits we display and inherit. That’s because at first no one had any idea that DNA was really all that important. People just assumed it was yet another cellular substance in a deluge of substances being discovered during that period. However, at roughly the same time that Miescher was doing his work, other scientists were finding new ways to observe never before seen structures and processes in cells. But it was a long time before anyone suspected that these cellular observations were related to DNA. This is a recurring theme in science: researchers in different fields find themselves studying different aspects of the same phenomenon, and it often takes decades for scientists to put all the pieces together to give an accurate context for all of their data.
If you think back to our original example about blue eyes, you might remember this biochemical chain of events:
Specific HERC2 gene in our DNA → rare HERC2 protein → fewer P proteins → less tyrosine → less melanin → bluer eyes
One thing I haven’t really discussed yet in this chain is the concept of a gene. We have an intuitive sense of what a gene is–it’s something that determines the presence or absence of certain of our traits–and we know that genes can be passed from parent to child. This was about the level of understanding of genes and heredity that scientists in the mid-1800s had. But this state of affairs was changing rapidly, and we’re going to talk about exactly how it was changing.
In the 1870’s, a German anatomist by the name of Walther Flemming was studying cells taken from the gills of salamanders (scientists are weird sometimes). He began introducing various dyes into his experiments to make it easier to see the cells’ internal structures under a microscope. Since the dyes didn’t kill the cells, he could watch them carry out various cellular duties in real time. During the course of these experiments, he noticed some threadlike structures inside the nucleus that were dyed very strongly, but that only really appeared when the cell was about to divide into two. Flemming also noticed that these threads seemed to multiply in number right before they divided. In fact, it looked as though the threads precisely doubled before the cell split. He referred to the colored substance as chromatin, from the Greek for “colored,” and dubbed the overall cellular process of producing more threads and dividing as mitosis, from the Greek for “thread.” Later another scientist would begin referring to individual threads as chromosomes, Greek (again) for “colored bodies.” Here’s a sample of Flemming’s own drawings of what he observed:
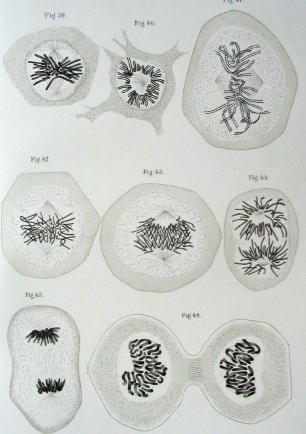
Around the same time, a Belgian named Edouard van Beneden was doing similar experiments on the eggs of parasitic roundworms (did I mention that scientists are weird sometimes?). In a 125-page tour de force, he wrote up his results, but not before noticing subtle (but, unbeknownst to him, important) differences between his results and those of Flemming. Van Beneden had been preserving his specimens in alcohol, but he had essentially ignored one of these specimens for several months. By sheer luck, it turns out that alcohol penetrates the layers of roundworms exceedingly slowly. When van Beneden went back to look at these old samples, he noticed that as the alcohol had seeped into the worms, it had stopped the eggs at successive points along their development, so that he basically could observe a slideshow of events in egg development.
The most important observation that van Beneden made in this paper was that the eggs seemed to divide before their chromosomes doubled. Flemming had established that in mitosis, the chromosomes doubled and then the cell split. Van Beneden was observing that the cell split, then the chromosomes doubled, then the cell split again! We call this process meiosis, which means “lessening” in Greek. The discovery of meiosis was important because van Beneden and others had also observed that no chromosomes disappeared when a sperm joined with an egg. That meant that a fertilized egg had double the number of chromosomes than an unfertilized egg. But all the rest of the cells in the body had the same number of chromosomes as the fertilized egg, so the question of how it was that unfertilized egg and sperm cells have half that number was unanswered until van Beneden’s discovery of meiosis.
The discovery of different pathways of cell division, mitosis and meiosis, might have all just been academic were it not for the work of a scientist named August Weismann. Weismann was intrigued by the observation that all the cells in an organism’s body except for sperm and egg cells seemed to have an even number of chromosomes–to be more precise, each chromosome seemed to be a member of a pair. So in humans, for example, most cells have 46 chromosomes, but they’re really laid out as 23 pairs of chromosomes. (These cells are known as somatic cells). Furthermore, in order for those cells to split, the 46 chromosomes have to double to 92, and each daughter cell then receives half of them. This was Flemming’s mitosis at work. However, in sperm and egg cell (or gametic cells, as they’re known) development, a progenitor cell having 46 chromosomes (23 pairs) would split such that each daughter cell only received one member of the pair, giving the daughter cells 23 chromosomes each. This was van Beneden’s meiosis. Van Beneden also observed that when a sperm and an egg come together during fertilization, the chromosomes from each could pair up again to give normal somatic cells. So if the sperm and egg were human, each would have 23 chromosomes which would then pair up to give the 46 chromosomes that we observe in all human somatic cells. To Weismann, this meant that meiosis is basically just the fertilization process in reverse. So he put forth the theory that heredity, the passing down of traits from parent to child, can only be mediated by whatever is contained in the sperm and egg. He believed that there was a fundamental difference between gametic cells and somatic cells, and that only gametic cells were involved in the passing of hereditary information.
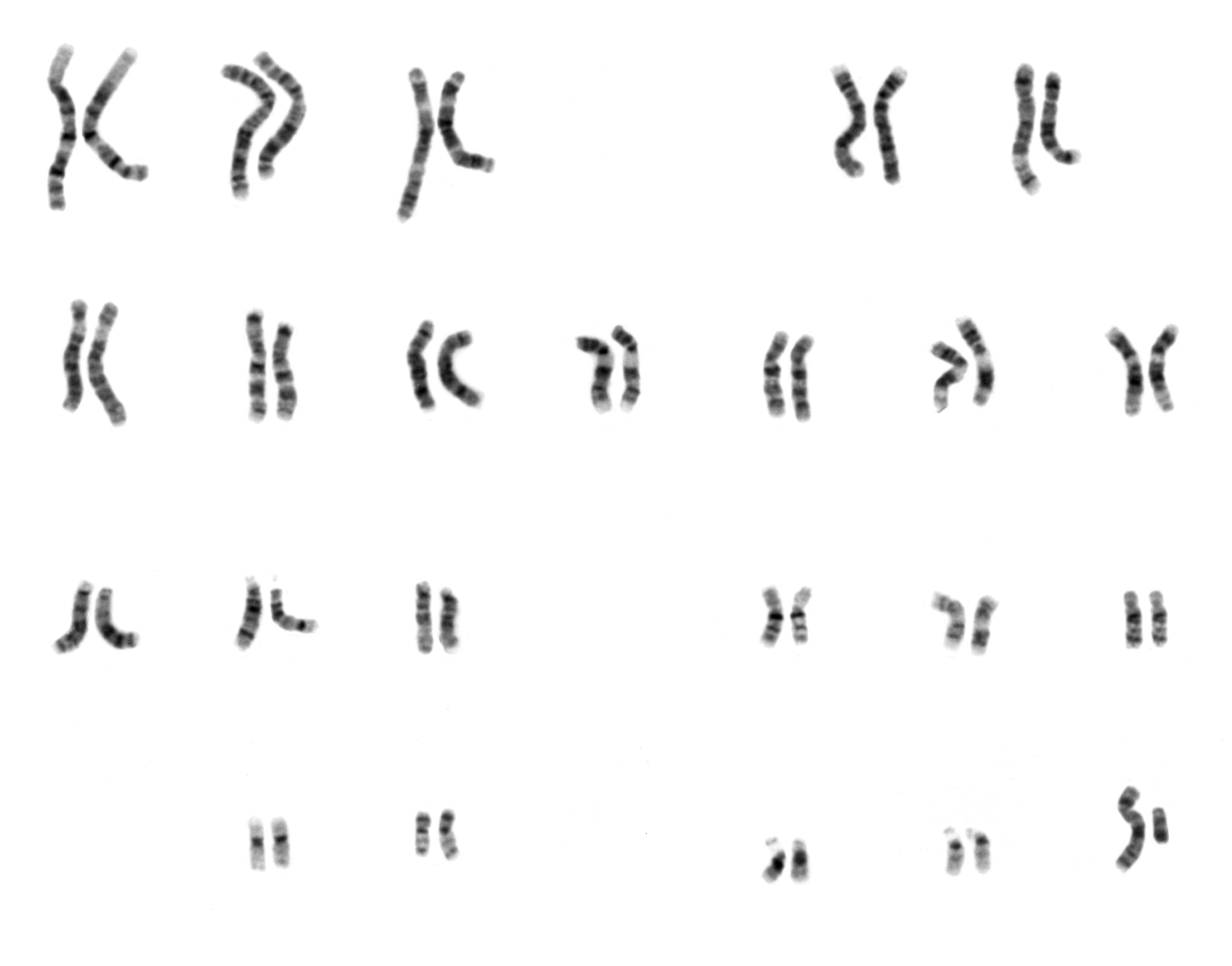
Weismann’s theory made a controversial prediction about inheritance. If offspring only get genetic information from the gametic cells (sperm and egg), and if gametic cells are the only cells in the body that are produced via meiosis, then the other cells in the body did not contribute any genetic information to the offspring. This may seem like a minor point, but it’s vitally important. What it means is that characteristics acquired by a parent in her lifetime do not get passed on to her offspring. So for example, if I’m a bodybuilder and I work out until I have really big muscles, it doesn’t automatically mean my kids will also have really big muscles. Likewise, Weismann illustrated this prediction by showing that mice whose tails had been removed had no increased chance of giving birth to offspring without tails.
It might seem obvious to us that the characteristics we work so hard to build up (or the various injuries and battle scars of life we accumulate) don’t necessarily get passed on to our children. But in Weismann’s time, it was widely believed that acquired traits could be passed on: in fact, that was the mechanism by which evolution was supposed to have taken place. In probably the most famous example, it was argued that giraffes evolved to have long necks because successive generations of giraffes had to keep straining and stretching their necks to reach food on higher and higher tree branches. But if Weismann was right and acquired traits weren’t passed on, then what mechanism could possibly account for such strange adaptations as the giraffe’s long neck? I don’t have nearly enough space here to get into the all the nitty gritty details regarding evolutionary science (don’t worry; I’ll do so in a later HDWKI), but I did want to point out that this aspect of Weismann’s theory actually caused quite an uproar.
Because of the controversy over whether or not acquired traits could be inherited, Weismann’s work didn’t really see its proper appreciation until the discovery (or rather re-discovery) of the mathematical laws of inheritance. The story goes that Gregor Mendel, a German monk and botanist, observed thousands of pea plants in the garden of the monastery where he lived, making note of traits such as flower color and plant height from generation to generation. (As a brief personal aside: research science can be quite boring sometimes–waiting for experiments to finish, sifting through data trying to make sense of it, tweaking and re-tweaking equipment to pull more data out of it. But every once in a while, as a scientist, I read a description of someone else’s research that makes me think, “Man, that just sounds mind-numbingly boring.” In the discovery of the laws of inheritance, I’ve gotta say I’m not surprised at all that these discoveries came from a monastery first. Given all of the exciting things going on in science in the mid-1800’s, if someone had come to me and said, “Hey, how would you like to watch thousands of pea plants grow for the next decade or so?” I’d have had to pass.) Mendel noted that offspring of the same parent pea plants displayed traits in very precise ratios. Let’s look at the colors of the pea plants’ flowers, for example. Pea flowers are either white or purple:
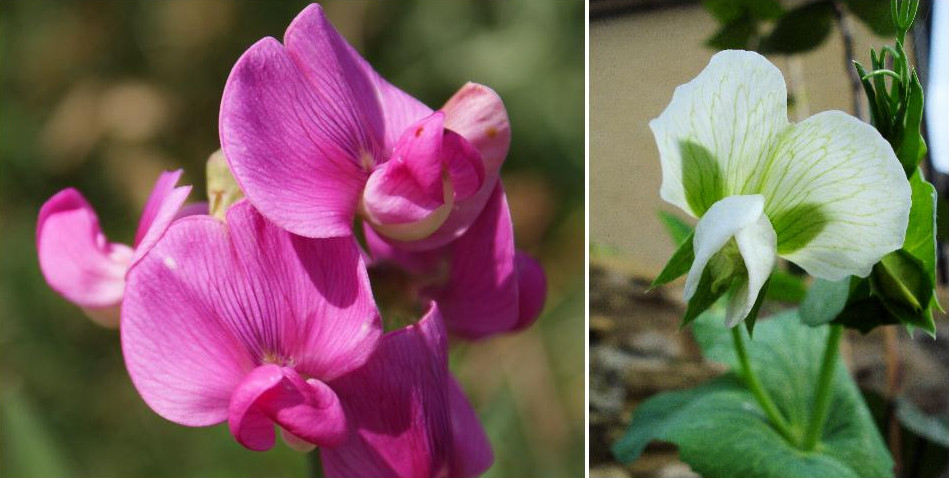
Mendel noticed that crossbreeding white-flowered plants with white-flowered plants always gave offspring with white flowers. However, this wasn’t always the case with purple-flowered plants. Most of the time, the offspring were purple-flowered, but every once in a while, a white-flowered offspring would appear. Mendel showed that the purple-flowered plants could always be separated into two groups by crossbreeding them exclusively with white-flowered plants. Some purple-flowered plants only produced purple-flowered offspring, regardless of whether they were crossbred with purple or white plants. Other purple-flowered plants, when crossed with white plants, produced purple or white-flowered offspring in about a 50-50 ratio of purple to white. Mendel’s real breakthrough came when he looked at the grandchildren: the offspring of the offspring. First, he isolated some purple-flowered grandparents that only produced purple offspring. Then he crossed them with white-flowered grandparents. All of their children were purple-flowered, as we just mentioned. However, he then took the offspring and crossed them with white-flowered plants. The result of this breeding was always 50-50 purple to white flowers for the grandchildren. Furthermore, if he crossed the purple offspring with each other, he always got a 3:1 ratio of purple to white-flowered plants.
What’s going on here? Well, Mendel was a pretty clever guy. He proposed that there were two variations of the flower color gene: purple and white. He also proposed that each individual pea plant has two copies of that gene. Since there are two possible colors, each plant can either have two copies of purple genes, two copies of white genes, or one copy each of purple and white. He theorized that the parents supply one copy of these genes at random to their offspring. So if a parent has one purple and one white gene, the offspring has a 50-50 chance of getting a purple or a white gene from that parent. If the other parent has two white genes, then the offspring will only be able to get a white gene from that parent. This means that the offspring will either have one purple and one white gene or two white genes. At this point, it’s probably best for me to just accept that a picture is worth a thousand words. Let’s pretend both parents have one purple and one white gene. We can make a diagram like this:
Mom has one purple gene and one white gene, as seen on the top row. So does Dad, as we see in the column to the left. This means 1/4 of the kids will get two purple genes, and 1/4 of the kids will get two white genes, while the remaining half of the kids will get one gene of each color. But what does this mean for the actual color of the flowers? After all, the flowers are either purple or white. There’s no half-purple, half-white flower.
Mendel proposed that one of the genes was dominant over the other. In this case, the purple color was dominant over the white color. So if you’re one of the kids that had one purple and one white gene, congratulations: you have purple flowers. The only time white flowers would show up is if both of your genes were white. Mendel called this type of gene “recessive.” We can actually go back and recreate Mendel’s experimental results using diagrams like the one above (these are called Punnett squares, after their inventor Reginald Punnett).
So let’s do the experiment (on paper, at least. I don’t have a decade or thousands of pea plants to reproduce the experiment for real). Remember, Mendel started with purple-flowered plants that never seemed to produce white-flowered offspring. Based on his theory, these purple-flowered plants most likely had two copies of purple genes. He then bred them with white-flowered plants. Remember again that plants only had white flowers if they had two copies of white genes. So let’s say the mom had two white genes and the dad had two purple genes. What would the kids look like?
We can see that if the mom had two white genes and the dad had two purple genes, then the kids would each have one white and one purple gene. Since the purple gene is dominant, the kids would all have purple flowers, as Mendel predicted. But now if we take one of the kids and breed it with another white-flowered plant:
We see that half the kids have one purple and one white gene, and half the kids have two white genes. This means that half the kids will have purple flowers and half the kids will have white flowers. This is where the predicted 50-50 ratio comes from. But what if we did the other experiment, where we crossbreed the two kids?
Turns out it’s the same diagram as the first one we looked at. A quarter of the kids will have two purple genes, and a quarter will have two white genes, and the remaining half will have one purple and one white. But since purple is dominant over white, 3/4 of the kids will have purple flowers and 1/4 of the kids will have white flowers. This is where the 3:1 ratio comes from, exactly as Mendel observed with his pea plants.
We initially mentioned that Mendel’s work was crucial in the acceptance of Weismann’s theory. It’s pretty clear why. Weismann insisted that all of the genetic information that an offspring receives comes through the sperm and egg cells, and van Beneden had observed that sperm and egg each contribute one of a pair of chromosomes. Mendel, knowing nothing about chromosomes or meiosis, noted that his observations on inheritance in peas only worked if each individual offspring received one of a pair of genes from its parents. It seems that Weismann’s chromosomes are the perfect vehicle to ferry Mendel’s genes from parent to child, but unfortunately, Mendel’s work (published in 1865-66) was unknown to Weismann (whose work was published in the 1880’s), and Mendel was largely forgotten until about 1900, when his writings were rediscovered. Scientists then quickly pieced together that genes and chromosomes were inextricably linked, but it was too late for Mendel. He had died in 1884 as the humble author of a few barely-known papers on pea plant hybrids, never realizing the profound impact his studies would have on our understanding of the most fundamental aspects of life itself.
Well, that was kind of a downer. But it turns out that when Mendel’s work eventually was rediscovered, it propelled the science of genetics forward by leaps and bounds, exciting great interest that we may be growing ever closer to the molecular nature of life. Join us next time when we discuss what happened during this period, and how it led us closer to cracking the genetic code.
References
(Some of) Flemming’s work can be found here, in its original German:
A history of van Beneden and his discovery of meiosis can be found here (Just click on the “Full Text (PDF)” tab):
August Weismann’s germ plasm theory is described in his textbook and several collected essays, here:
- http://www.esp.org/books/weismann/germ-plasm/facsimile/
- http://www.esp.org/books/weismann/essays/facsimile/
A great pop-sci account of Mendel’s work is given in the book:
-
The Monk in the Garden: The Lost and Found Genius of Gregor Mendel, the Father of Genetics, by Robin Marantz Henig.